Integrating stress signals—XBP1 as a novel mediator of intercellular crosstalk in non-alcoholic steatohepatitis
Non-alcoholic steatohepatitis (NASH), the most advanced form of non-alcoholic fatty liver disease (NAFLD), is the most common chronic liver disease and will soon be the most common reason for liver transplantation as NASH has no approved pharmacotherapy. This unmet need is due in part to an incomplete understanding of mechanistic determinants of various aspects of NASH pathogenesis, and uncertain progression between a prevalent pre-NASH state (lipid accumulation) to the hepatocyte injury, inflammation and fibrosis that defines NASH (1).
NASH develops in the context of obesity, but people of similar body weight and adiposity do not have identical risk of NASH development. This discordance highlights a genetic component to the disease, well-established for hepatic lipid accumulation from genome-wide association studies (GWAS), but less so for liver inflammation and fibrosis. One possibility is that heterogeneity in NASH arises from altered transcriptional regulation that may not be easily uncovered by GWAS, as multiple metabolic and developmental signaling pathways are preferentially dysregulated in NASH but not pre-NASH states (2). These and other studies have suggested that two or more “hits” are necessary for NASH development (3,4). In this conceptual framework, lipid accumulation in hepatocytes, termed steatosis, may (but not necessarily) prompt a second hit, for example, oxidative stress, which will trigger hepatocyte death, immune cell activation, and eventually, the deposition of collagen fibrils by activated hepatic stellate cells (HSCs). Other work suggests that NASH development is augmented by extrahepatic mechanisms, including gut- and adipose tissue-derived proinflammatory factors, which may be synergetic. In fact, inflammation may theoretically precede or even provoke steatosis.
Chronic endoplasmic reticulum (ER) stress is linked to pathogenesis of multiple diseases (5-7), including obesity-induced metabolic diseases such as type 2 diabetes (T2D) and NASH, suggesting a possible pathogenic role. ER stress is often associated with the accumulation of unfolded proteins in the ER, which bind to the binding immunoglobulin protein (BIP), and triggers three, parallel signaling pathways—inositol-requiring enzyme 1α (IRE1α), which activates c-Jun NH2-terminal kinase (JNK), causing apoptosis, and induces splicing of X-box binding protein 1 (XBP1), leading to transcriptional upregulation of genes involved in lipogenesis, folding and ER-associated degradation (ERAD); PKR-like ER kinase (PERK)-induced phosphorylation of eukaryotic translation initiation factor 2α (eIF2α), which leads to increased activating transcription factor (ATF4), and upregulated gene expression associated with amino acid metabolism, anti-oxidative stress and apoptosis; and activating transcription factor 6α (ATF6α), which is further processed to release a transcriptionally active cytoplasmic domain, leading to increase of ERAD and protein folding associated gene expression (5). Collectively, these signaling pathways are thought to alleviate ER stress through multiple mechanisms, including degradation of unfolded proteins, inhibition of new translation, and increase in ER capacity, which collectively augment likelihood of cellular survival. In chronic, unresolved ER stress, apoptosis and cell death ensue (5,8).
Multiple prior studies have evaluated whether ER stress pathways are altered in liver disease (9,10). In patients with NASH, for example, several groups have shown heterogeneous activation of liver ER stress signaling pathways, with some patients showing a p-JNK/p-eIF2α activity signature while others showing increased spliced XBP1 (11-13). This led to studies to test potential for causality using genetically-modified mouse models, with somewhat mixed results. Constitutive and systemic deletion of ATF6 provoked excess lipid accumulation in liver (14). On the other hand, ATF4 knockout mice were protected from high-fat diet (HFD) induced steatosis (15,16). Similarly, inducible full knockout XBP1 or hepatocyte-specific XBP1 knockout mice demonstrated reduced de novo lipogenesis, leading to relative protection from steatosis (17,18). Genetic deletion of B-cell lymphoma 2 (BCL2)-associated X protein (Bax) inhibitor-1 (BI-1), a suppressor of IRE1α, induced ER stress and hepatic NOD-like receptor family, pyrin domain containing 3 (NLRP3) inflammasome activation, leading to hepatocyte death, inflammation and fibrosis (19). These data suggest that although often lumped as ER stress pathways, differential activation may lead to altered disease pathogenesis.
However, most work thus far has been on mouse models of hepatocyte-specific manipulation or systemic deletion of these key regulators. As such, whether these signals also regulate behavior of liver non-parenchymal cells (NPCs) that define NASH pathogenesis in vivo is less well-understood. To address this question, in a recent issue of Journal of Hepatology, Wang et al. showed increased mRNA expression of spliced XBP1 (XBP1s) in patients with as compared to without NASH (20). This led to a significant increase in XBP1s protein in whole liver, and a dramatic increase in both hepatocyte and NPC XBP1s, especially macrophages. Next, the authors generated cell-type-specific XBP1 knockout mice to determine if increase in XBP1s in hepatocytes, myeloid cells or both may contribute to liver injury induced by long-term HFD-feeding or methionine-choline deficient (MCD) diet-feeding. This particular choice of modeling was perhaps intentionally complementary. HFD-feeding mimics pre-NASH phenotypes, causing profound obesity (and subsequent insulin resistance → increased de novo lipogenesis → steatosis), but only mild inflammation and fibrosis. MCD-feeding is a more toxic liver injury, leading to rapid hepatocyte death, liver inflammation and fibrosis that pathologically resembles human NASH, despite progressive weight loss that does not mimic the human condition. But intriguingly, hepatocyte-specific XBP1 knockout mice were protected from both HFD- and MCD-induced hepatic steatosis, attributed to inhibition of de novo lipogenesis and increased lipid oxidation, consistent with prior studies using other XBP1-deficient mouse models (17,18).
The authors simultaneously generated myeloid-specific XBP1 knockout mice, which were similarly protected from both HFD- and MCD-induced hepatic lipid accumulation. Intriguingly, both hepatocyte and myeloid-specific XBP1 knockout mice gained less weight than control mice when fed HFD, resulting in less visceral fat. Food intake was reportedly unchanged, suggesting increased energy expenditure, but this was not experimentally verified. The mechanism attributed to this finding was altered macrophage polarization, as knockout animals showed a shift in liver macrophage populations, with a decrease in so-called M1 (or classically activated) and increased M2 (alternatively activated) macrophages. This shift was accompanied by a decrease in NLRP3 inflammasome levels, which in turn reduced expression of multiple inflammatory markers and, through a somewhat less clear mechanism, inhibited hepatocyte lipogenesis. Notably, the M1/M2 ratio proposed to explain differential inflammatory nature of tissue macrophage populations may be less revealing than originally thought. For example, patients with NASH show higher levels of both M1 and M2 macrophage marker genes, suggesting that after liver injury, a subset of M2 macrophages may be involved in tissue remodeling, which may increase the risk of fibrosis (21,22). Indeed, in this study, the authors sorted peripheral blood mononuclear cells in NASH patients and found increase of both M1 and M2 macrophage populations, and a similar increase in livers of NASH patients. More refined evaluation of these cells, for instance by fluorescence-activated cell sorting (FACS) or single-cell RNA (scRNA) sequencing, may help determine impact of XBP1 in macrophage biology, in particular to help determine XBP1 expression in different myeloid populations in patients with NASH.
Collectively, results from Wang et al. suggest that activation of XBP1 in hepatocytes and myeloid cells cooperatively determine multiple aspects of NAFLD/NASH pathology (Figure 1). Simultaneously, or perhaps as a result, liver fibrosis was decreased, postulated by the authors as due to reduced TGFβ signaling to HSCs, the cell type responsive for collagen deposition in response to liver injury (23). These mouse data are on the backdrop of a clear increase in XBP1 expression in patients with NASH patients, which was associated with markers of liver injury and lipogenic/proinflammatory gene expression. A striking finding of this study is that XBP1high patients have more liver fibrosis, which is the major determinant of morbidity and mortality in patients with NASH. This data may reconcile uneven associations between XBP1 and patient characteristics in other studies (11-13), if as described, XBP1 levels correlate with NASH severity. Further patient cohorts may be helpful to clarify these associations.
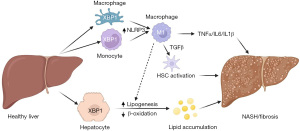
Mechanism of higher XBP1 expression in these cell types is not known. For example, activation of toll-like receptors (TLRs) induced XBP1 expression even in the absence of ER stress (24), notable as deficiency of hepatocyte TLR4 markedly reduced liver fibrosis in mice (25). Future work is necessary to determine if other ER stress pathways in myeloid cells lead to similar phenotypes, and/or if XBP1 represents a new gut-liver axis. The potential translational relevance of this finding may be quite significant. For example, authors used two chemical inhibitors (toyocamycin and TUDCA) that have been reported to inhibit XBP1 in HFD fed mice, both of which reduced liver injury, lipid accumulation and a-SMA staining, and decreased ratio of M1/M2 macrophage marker gene expression. Although both of these chemical compounds have been reported to inhibit other pathways as well, the studies presented here may provide impetus to generate specific XBP1 inhibitors that may have application for NAFLD/NASH.
Acknowledgments
Funding: None.
Footnote
Provenance and Peer Review: This article was commissioned by the editorial office, Translational Gastroenterology and Hepatology. The article did not undergo external peer review.
Conflicts of Interest: Both authors have completed the ICMJE uniform disclosure form (available at https://tgh.amegroups.com/article/view/10.21037/tgh-22-73/coif). The authors have no conflicts of interest to declare.
Ethical Statement: The authors are accountable for all aspects of the work in ensuring that questions related to the accuracy or integrity of any part of the work are appropriately investigated and resolved.
Open Access Statement: This is an Open Access article distributed in accordance with the Creative Commons Attribution-NonCommercial-NoDerivs 4.0 International License (CC BY-NC-ND 4.0), which permits the non-commercial replication and distribution of the article with the strict proviso that no changes or edits are made and the original work is properly cited (including links to both the formal publication through the relevant DOI and the license). See: https://creativecommons.org/licenses/by-nc-nd/4.0/.
References
- Huby T, Gautier EL. Immune cell-mediated features of non-alcoholic steatohepatitis. Nat Rev Immunol 2022;22:429-43. [Crossref] [PubMed]
- Steinman JB, Salomao MA, Pajvani UB. Zonation in NASH - A key paradigm for understanding pathophysiology and clinical outcomes. Liver Int 2021;41:2534-46. [Crossref] [PubMed]
- Day CP, James OF. Steatohepatitis: a tale of two "hits"? Gastroenterology 1998;114:842-5. [Crossref] [PubMed]
- Tilg H, Moschen AR. Evolution of inflammation in nonalcoholic fatty liver disease: the multiple parallel hits hypothesis. Hepatology 2010;52:1836-46. [Crossref] [PubMed]
- Hetz C. The unfolded protein response: controlling cell fate decisions under ER stress and beyond. Nat Rev Mol Cell Biol 2012;13:89-102. [Crossref] [PubMed]
- Ozcan U, Cao Q, Yilmaz E, et al. Endoplasmic reticulum stress links obesity, insulin action, and type 2 diabetes. Science 2004;306:457-61. [Crossref] [PubMed]
- Batista A, Rodvold JJ, Xian S, et al. IRE1alpha regulates macrophage polarization, PD-L1 expression, and tumor survival. PLoS Biol 2020;18:e3000687. [Crossref] [PubMed]
- Grootjans J, Kaser A, Kaufman RJ, et al. The unfolded protein response in immunity and inflammation. Nat Rev Immunol 2016;16:469-84. [Crossref] [PubMed]
- Jo H, Choe SS, Shin KC, et al. Endoplasmic reticulum stress induces hepatic steatosis via increased expression of the hepatic very low-density lipoprotein receptor. Hepatology 2013;57:1366-77. [Crossref] [PubMed]
- Lebeaupin C, Vallée D, Hazari Y, et al. Endoplasmic reticulum stress signalling and the pathogenesis of non-alcoholic fatty liver disease. J Hepatol 2018;69:927-47. [Crossref] [PubMed]
- Puri P, Mirshahi F, Cheung O, et al. Activation and dysregulation of the unfolded protein response in nonalcoholic fatty liver disease. Gastroenterology 2008;134:568-76. [Crossref] [PubMed]
- González-Rodríguez A, Mayoral R, Agra N, et al. Impaired autophagic flux is associated with increased endoplasmic reticulum stress during the development of NAFLD. Cell Death Dis 2014;5:e1179. [Crossref] [PubMed]
- Lake AD, Novak P, Hardwick RN, et al. The adaptive endoplasmic reticulum stress response to lipotoxicity in progressive human nonalcoholic fatty liver disease. Toxicol Sci 2014;137:26-35. [Crossref] [PubMed]
- Rutkowski DT, Wu J, Back SH, et al. UPR pathways combine to prevent hepatic steatosis caused by ER stress-mediated suppression of transcriptional master regulators. Dev Cell 2008;15:829-40. [Crossref] [PubMed]
- Xiao G, Zhang T, Yu S, et al. ATF4 protein deficiency protects against high fructose-induced hypertriglyceridemia in mice. J Biol Chem 2013;288:25350-61. [Crossref] [PubMed]
- Seo J, Fortuno ES 3rd, Suh JM, et al. Atf4 regulates obesity, glucose homeostasis, and energy expenditure. Diabetes 2009;58:2565-73. [Crossref] [PubMed]
- Lee AH, Scapa EF, Cohen DE, et al. Regulation of hepatic lipogenesis by the transcription factor XBP1. Science 2008;320:1492-6. [Crossref] [PubMed]
- So JS, Hur KY, Tarrio M, et al. Silencing of lipid metabolism genes through IRE1alpha-mediated mRNA decay lowers plasma lipids in mice. Cell Metab 2012;16:487-99. [Crossref] [PubMed]
- Lebeaupin C, Vallée D, Rousseau D, et al. Bax inhibitor-1 protects from nonalcoholic steatohepatitis by limiting inositol-requiring enzyme 1 alpha signaling in mice. Hepatology 2018;68:515-32. [Crossref] [PubMed]
- Wang Q, Zhou H, Bu Q, et al. Role of XBP1 in regulating the progression of non-alcoholic steatohepatitis. J Hepatol 2022;77:312-25. [Crossref] [PubMed]
- Rensen SS, Slaats Y, Nijhuis J, et al. Increased hepatic myeloperoxidase activity in obese subjects with nonalcoholic steatohepatitis. Am J Pathol 2009;175:1473-82. [Crossref] [PubMed]
- Kazankov K, Jørgensen SMD, Thomsen KL, et al. The role of macrophages in nonalcoholic fatty liver disease and nonalcoholic steatohepatitis. Nat Rev Gastroenterol Hepatol 2019;16:145-59. [Crossref] [PubMed]
- Schwabe RF, Tabas I, Pajvani UB. Mechanisms of Fibrosis Development in Nonalcoholic Steatohepatitis. Gastroenterology 2020;158:1913-28. [Crossref] [PubMed]
- Martinon F, Chen X, Lee AH, et al. TLR activation of the transcription factor XBP1 regulates innate immune responses in macrophages. Nat Immunol 2010;11:411-8. [Crossref] [PubMed]
- Yu J, Zhu C, Wang X, et al. Hepatocyte TLR4 triggers inter-hepatocyte Jagged1/Notch signaling to determine NASH-induced fibrosis. Sci Transl Med 2021;13:eabe1692.
Cite this article as: Yu J, Pajvani UB. Integrating stress signals—XBP1 as a novel mediator of intercellular crosstalk in non-alcoholic steatohepatitis. Transl Gastroenterol Hepatol 2023;8:13.