Unboxing the cell-type specific contribution of endoplasmic reticulum stress to NASH pathophysiology—myeloid X-box-binding protein 1 as a driver of steatohepatitis and fibrosis
Non-alcoholic steatohepatitis (NASH) is characterised by hepatocellular lipid accumulation, lipotoxicity-mediated hepatocellular injury and subsequent liver inflammation (1). One cell population particularly involved in NASH pathogenesis are liver macrophages, i.e., liver-resident Kupffer cells and monocyte-derived macrophages. Liver macrophages are recognised as central regulators of the hepatic microenvironment, and under healthy conditions they display a restorative, immune-suppressive phenotype to uphold homeostasis. However, they acquire an inflammatory phenotype upon metabolic stress and subsequent hepatocellular injury induced by increased abundance of free fatty acids and insulin resistance in NASH. In these conditions, macrophages release inflammatory cytokines like tumour necrosis factor alpha (TNF-α) and interleukin-1 beta (IL-1β), which can perpetuate hepatocellular injury by providing feedback signals to hepatocytes. Furthermore, macrophage-derived transforming growth factor beta (TGF-β) drives fibrogenesis through the activation and trans-differentiation of hepatic stellate cells into myofibroblasts (2). In a significant proportion of patients, these processes ultimately lead to liver fibrosis and its clinically relevant outcomes of liver failure or hepatocellular carcinoma.
One central mechanism linking hepatocellular steatosis and the hepatic inflammatory response is endoplasmic reticulum (ER) stress in hepatocytes. In NASH, ER function is impaired by oxidative stress, accumulation of free fatty acids, hyperglycaemia and subsequent lipotoxicity, resulting in the accumulation of unfolded proteins. These unfolded proteins, in turn, activate conserved sensors of the unfolded protein response (UPR), namely activating transcription factor 6 (ATF-6), protein kinase RNA-like endoplasmic reticulum kinase (PERK) and inositol-requiring-enzyme 1 alpha (IRE1α), which instigate the ER stress response via distinct signalling pathways (3). IRE1α is highly conserved and has dual enzymatic activity: it activates JNK and NFκB through its serine/threonine kinase, and the endoribonuclease activity digests and inactivates mRNAs and miRNAs, in a process coined regulated IRE1α-dependent decay (RIDD). IRE1α endoribonuclease also targets the mRNA of the UPR regulator X-box binding protein-1 (XBP1) and catalyses the unconventional splicing to the transcriptionally active form XBP1s. XBP1s then acts as a transcription factor and enhances gene expression related to protein folding, lipid synthesis and ER-associated degradation (ERAD). First and foremost, ER stress is a physiologic adaptive cellular stress response. It aims to restore ER function by reducing protein translation and upregulating ER protein folding capacity, autophagy and the ERAD pathway. However, prolonged and overwhelming activation of ER stress plays a role in the development of many diseases, including cancer, type 2 diabetes and the metabolic syndrome (4). In NASH, ER stress drives hepatocellular de novo lipogenesis, lipid accumulation and disturbed cholesterol metabolism. Upon overwhelming cellular stress, it promotes hepatocellular injury and liver inflammation via activating pro-apoptotic signalling pathways and RIDD (3). Pharmacological inhibition of IRE1α endoribonuclease activity or Caspase-2 downstream of IRE1α has shown beneficial effects in transgenic, ER-stress and high-fat diet (HFD)-induced pre-clinical models of NASH, resulting in reduced lipid accumulation and liver inflammation (5,6). ER stress, and the IRE1α-XBP1 axis in particular, are directly involved in pro-inflammatory activation of macrophages (4), but the role of myeloid XBP1 in non-alcoholic fatty liver disease (NAFLD) and NASH had not been studied.
In their recent paper in the Journal of Hepatology, Wang and colleagues provided a novel angle on the role of XBP1 in NASH (Figure 1). They found increased expression of XBP1s in human liver macrophages and hepatocytes in NASH and hypothesised that myeloid XBP1 is important for shaping liver macrophage function, hepatic lipid metabolism, inflammation and fibrosis in NASH (7). To study the role of macrophage XBP1, they generated myeloid-specific XBP1 knockout (XBP1ΔMf) mice and subjected these to HFD and methionine-choline-deficient diet (MCD) models of NASH. In both HFD and MCD, macrophage XBP1 deficiency attenuated lipid accumulation, liver inflammation and fibrosis, and improvements in liver histology were accompanied by a shift of liver macrophage polarisation towards a more restorative phenotype. These findings agree with previous reports that the IRE1α-XBP1 pathway drives inflammatory macrophage polarisation (8). However, how the XBP1 knockout prevents sustained inflammatory activation of liver macrophages remained obscure. Changes in macrophage function are characterised by shifts in immune cell metabolism, and lipogenic pathways are prominently regulated by IRE1α-XBP1. Thus, it would be interesting to study how XBP1 knockout affects macrophage metabolism and how this translates into altered cell function. Furthermore, the authors present the changes in macrophage polarisation as a clear-cut switch from ‘M1’ to ‘M2’. However, such “black-or-white” macrophage phenotypes (M1 vs. M2) do not exist in the liver or in NASH (9). The complex biology of macrophages warrants further detailed investigation, and single-cell technologies offer the opportunity to gain insights into the heterogeneity of such functional changes and get a picture of the overall immune landscape in response to myeloid XBP1 deletion (10).
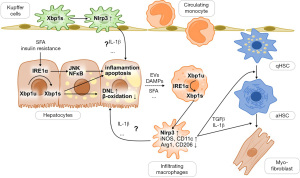
ER stress and its IRE1α-XBP1 branch are potent activators of the Nlrp3 inflammasome (11,12), and indeed, XBP1ΔMf resulted in dampened hepatic Nlrp3 inflammasome activity. Using myeloid-specific knockout and lentiviral overexpression of Nlrp3, Wang et al. (7) showed that Nlrp3 links XBP1s to hepatocellular injury. In primary hepatocytes co-cultured with wild-type bone-marrow- or liver-derived macrophages, palmitate treatment increased lipogenic gene expression and decreased the expression of beta-oxidative genes, leading to a net lipid accumulation. This effect on lipid metabolism was abrogated by replacing wild-type with XBP1ΔMf or Nlrp3ΔMf macrophages. Notably, lentiviral overexpression of Nlrp3 in XBP1ΔMf macrophages restored the changes in lipid metabolism to wild-type levels. This suggests that the Nlrp3 inflammasome mediates the effects of myeloid XBP1 on hepatocellular lipid metabolism (Figure 1). However, further investigation needs to address how exactly macrophage IRE1α-XBP1s-induced Nlrp3 mediates hepatocellular lipid accumulation. Nlrp3 activity leads to the release of macrophage-derived IL-1β, which is the major downstream effector cytokine of the Nlrp3 inflammasome and alters lipid metabolism in hepatocytes (13). Thus, IL-1β is a candidate for mediating macrophage XBP1s effects on hepatocellular steatosis.
Similar to the myeloid cell-specific knockout, improved hepatocellular lipid metabolism, inflammation and fibrosis were also seen in hepatocellular XBP1 knockout (XBP1Δhep) mice (7). Thus, cell-type specific XBP1 seems to have a non-redundant role in the development and perpetuation of NASH. In principle, this could make XBP1 an attractive therapeutic target, potentially affecting steatotic and inflammatory mechanisms in different cell types at the same time. However, ER stress is a physiologic response to cellular stress, and complete suppression of the IRE1α-XBP1 pathway might be harmful in a therapeutic setting, as indicated by previous studies that reported increased hepatocellular injury upon hepatocellular XBP1 knockout in fructose- or high-fat-high-fructose-feeding (14,15), thioacetamide-induced acute liver injury (16) and partial hepatectomy (17). Other studies observed similar effects for hepatocyte-specific deletion of IRE1α, which result in increased hepatocellular injury upon tunicamycin-induced ER stress (18) while attenuating liver injury in high-fat, high-fructose, high-cholesterol-induced murine NASH (19). These findings raise concerns of hepatotoxicity, and we need to understand better the mechanisms causing such deleterious effects to define the true potential of NASH treatments targeting IRE1α-XBP1s.
ER stress is a central regulator of cellular function not restricted to the liver, and consequently, myeloid XBP1 deletion led to extrahepatic effects. Therefore, lower body weight, visceral adipose tissue mass and attenuated visceral adipose tissue inflammation may well have contributed to the improved liver phenotype in XBP1ΔMf mice (7). While future studies need to address the relative contribution of liver-specific myeloid XBP1 in the development and progression of NASH, targeting ER stress in extrahepatic tissues might be beneficial. ER stress is involved in the pathogenesis of metabolic syndrome, type 2 diabetes and atherosclerosis (4), and these diseases are common comorbidities and significant determinants of all-cause mortality in patients with NASH. Therefore, blocking IRE1α could possibly target both the liver and metabolic disease. Conversely, transient and low-grade activation of IRE1α is an important adequate adaptive response to obesity (20), and any therapeutic strategies will need to avoid blocking the beneficial effects of ER stress.
In addition, IRE1α-XBP1 is critical for normal macrophage function and host response to bacterial infection (4), raising concerns of potential immunosuppressive side effects of IRE1α/XBP1 inhibition. Notably, increased rates of fatal infections were reported in the CANTOS trial, which tested an anti-IL-1β monoclonal antibody for treating patients with high cardiovascular risk (21). Additionally, such anti-inflammatory effects could impact tumour biology and theoretically increase the risk of (hepato-)carcinogenesis. However, immune cell IRE1α-XBP1 promotes the immunosuppressive cancer microenvironment and is generally recognised as a driver of tumour development and progression (22). Still, studies need to address the specific effects of IRE1α-XBP1 inhibition on hepatocarcinogenesis in NASH to ascertain the safety or even anti-tumour effectiveness of such a therapeutic approach.
Wang et al. addressed the need for novel therapeutic approaches by studying the effects of two different inhibitors of ER stress: toyocamycin as an experimental specific IRE1α endoribonuclease inhibitor and the chemical chaperone tauroursodeoxycholic acid (TUDCA). While the effectiveness of such treatments in transgenic ER-stress-induced models of NASH has been demonstrated before (5,6), the authors are the first to study the effects in a genuine diet-induced model. In agreement with existing evidence, they observed a reduction in transaminases, liver steatosis, inflammation and αSMA-positive area, and—consistent with an effect on myeloid ER stress—this was accompanied by a shift in macrophage polarisation towards iNOS−CD206+ cells (7). While IRE1α appears to represent an attractive therapeutic target, other components of the ER stress pathway are also worth consideration. Acetyl-CoA-carboxylase (ACC) and ketohexokinase (KHK) inhibitors do not target ER stress directly, but are in clinical testing already and potently inhibit de novo lipogenesis (23,24). Therefore, one can speculate that ACC and KHK inhibitors may alleviate ER stress through attenuated lipotoxicity, although this link is not fully established yet. Inhibition of Caspase-2 downstream of IRE1α-XBP1 reduces steatosis and inflammation in ER-stress-induced steatohepatitis (6), and other investigational compounds targeting ATF-6 and PERK branches of ER stress are available for pre-clinical testing and are discussed in detail elsewhere (3,25). The future will tell which, if any, approach to target ER stress is suitable for the treatment of NASH. Ideally, such a treatment would target liver disease and extrahepatic manifestations of the metabolic syndrome as well.
Acknowledgments
Funding: None.
Footnote
Provenance and Peer Review: This article was commissioned by the editorial office, Translational Gastroenterology and Hepatology. The article did not undergo external peer review.
Conflicts of Interest: Both authors have completed the ICMJE uniform disclosure form (available at https://tgh.amegroups.com/article/view/10.21037/tgh-22-65/coif). PH received funding from Novo Nordisk through the University of Birmingham, unrelated to the present manuscript. FT received grants from Allergan, BMS, Inventiva and Gilead to his institution; consultation fees from Allergan, Bayer, Gilead, BMS, Boehringer, Intercept, Ionis, Inventiva, Merz, Pfizer, Alnylam, NGM, CSL Behring, Novo Nordisk and Novartis; honoraria for lectures and presentations from Gilead, AbbVie, Falk, Merz and Intercept; payment for expert testimony from Alnylam; travel support from Gilead; and participated in the Pfizer advisory board. The authors have no other conflicts of interest to declare.
Ethical Statement: The authors are accountable for all aspects of the work in ensuring that questions related to the accuracy or integrity of any part of the work are appropriately investigated and resolved.
Open Access Statement: This is an Open Access article distributed in accordance with the Creative Commons Attribution-NonCommercial-NoDerivs 4.0 International License (CC BY-NC-ND 4.0), which permits the non-commercial replication and distribution of the article with the strict proviso that no changes or edits are made and the original work is properly cited (including links to both the formal publication through the relevant DOI and the license). See: https://creativecommons.org/licenses/by-nc-nd/4.0/.
References
- Peiseler M, Schwabe R, Hampe J, et al. Immune mechanisms linking metabolic injury to inflammation and fibrosis in fatty liver disease - novel insights into cellular communication circuits. J Hepatol 2022;77:1136-60. [Crossref] [PubMed]
- Lefere S, Tacke F. Macrophages in obesity and non-alcoholic fatty liver disease: Crosstalk with metabolism. JHEP Rep 2019;1:30-43. [Crossref] [PubMed]
- Lebeaupin C, Vallée D, Hazari Y, et al. Endoplasmic reticulum stress signalling and the pathogenesis of non-alcoholic fatty liver disease. J Hepatol 2018;69:927-47. [Crossref] [PubMed]
- Grootjans J, Kaser A, Kaufman RJ, et al. The unfolded protein response in immunity and inflammation. Nat Rev Immunol 2016;16:469-84. [Crossref] [PubMed]
- Lebeaupin C, Vallée D, Rousseau D, et al. Bax inhibitor-1 protects from nonalcoholic steatohepatitis by limiting inositol-requiring enzyme 1 alpha signaling in mice. Hepatology 2018;68:515-32. [Crossref] [PubMed]
- Kim JY, Garcia-Carbonell R, Yamachika S, et al. ER Stress Drives Lipogenesis and Steatohepatitis via Caspase-2 Activation of S1P. Cell 2018;175:133-45.e15. [Crossref] [PubMed]
- Wang Q, Zhou H, Bu Q, et al. Role of XBP1 in regulating the progression of non-alcoholic steatohepatitis. J Hepatol 2022;77:312-25. [Crossref] [PubMed]
- Martinon F, Chen X, Lee AH, et al. TLR activation of the transcription factor XBP1 regulates innate immune responses in macrophages. Nat Immunol 2010;11:411-8. [Crossref] [PubMed]
- Roohani S, Tacke F. Liver Injury and the Macrophage Issue: Molecular and Mechanistic Facts and Their Clinical Relevance. Int J Mol Sci 2021;22:7249. [Crossref] [PubMed]
- Ginhoux F, Yalin A, Dutertre CA, et al. Single-cell immunology: Past, present, and future. Immunity 2022;55:393-404. [Crossref] [PubMed]
- Robblee MM, Kim CC, Porter Abate J, et al. Saturated Fatty Acids Engage an IRE1α-Dependent Pathway to Activate the NLRP3 Inflammasome in Myeloid Cells. Cell Rep 2016;14:2611-23. [Crossref] [PubMed]
- Lebeaupin C, Proics E, de Bieville CH, et al. ER stress induces NLRP3 inflammasome activation and hepatocyte death. Cell Death Dis 2015;6:e1879. [Crossref] [PubMed]
- Stienstra R, Saudale F, Duval C, et al. Kupffer cells promote hepatic steatosis via interleukin-1beta-dependent suppression of peroxisome proliferator-activated receptor alpha activity. Hepatology 2010;51:511-22. [Crossref] [PubMed]
- Duwaerts CC, Siao K, Soon RK Jr, et al. Hepatocyte-specific deletion of XBP1 sensitizes mice to liver injury through hyperactivation of IRE1α. Cell Death Differ 2021;28:1455-65. [Crossref] [PubMed]
- Liu X, Henkel AS, LeCuyer BE, et al. Hepatocyte X-box binding protein 1 deficiency increases liver injury in mice fed a high-fat/sugar diet. Am J Physiol Gastrointest Liver Physiol 2015;309:G965-74. [Crossref] [PubMed]
- Liu Z, Wang M, Wang X, et al. XBP1 deficiency promotes hepatocyte pyroptosis by impairing mitophagy to activate mtDNA-cGAS-STING signaling in macrophages during acute liver injury. Redox Biol 2022;52:102305. [Crossref] [PubMed]
- Argemí J, Kress TR, Chang HCY, et al. X-box Binding Protein 1 Regulates Unfolded Protein, Acute-Phase, and DNA Damage Responses During Regeneration of Mouse Liver. Gastroenterology 2017;152:1203-16.e15. [Crossref] [PubMed]
- Zhang K, Wang S, Malhotra J, et al. The unfolded protein response transducer IRE1α prevents ER stress-induced hepatic steatosis. EMBO J 2011;30:1357-75. [Crossref] [PubMed]
- Dasgupta D, Nakao Y, Mauer AS, et al. IRE1A Stimulates Hepatocyte-Derived Extracellular Vesicles That Promote Inflammation in Mice With Steatohepatitis. Gastroenterology 2020;159:1487-503.e17. [Crossref] [PubMed]
- Madhavan A, Kok BP, Rius B, et al. Pharmacologic IRE1/XBP1s activation promotes systemic adaptive remodeling in obesity. Nat Commun 2022;13:608. [Crossref] [PubMed]
- Ridker PM, Everett BM, Thuren T, et al. Antiinflammatory Therapy with Canakinumab for Atherosclerotic Disease. N Engl J Med 2017;377:1119-31. [Crossref] [PubMed]
- Cubillos-Ruiz JR, Bettigole SE, Glimcher LH. Tumorigenic and Immunosuppressive Effects of Endoplasmic Reticulum Stress in Cancer. Cell 2017;168:692-706. [Crossref] [PubMed]
- Lally JSV, Ghoshal S, DePeralta DK, et al. Inhibition of Acetyl-CoA Carboxylase by Phosphorylation or the Inhibitor ND-654 Suppresses Lipogenesis and Hepatocellular Carcinoma. Cell Metab 2019;29:174-82.e5. [Crossref] [PubMed]
- Shepherd EL, Saborano R, Northall E, et al. Ketohexokinase inhibition improves NASH by reducing fructose-induced steatosis and fibrogenesis. JHEP Rep 2021;3:100217. [Crossref] [PubMed]
- Marciniak SJ, Chambers JE, Ron D. Pharmacological targeting of endoplasmic reticulum stress in disease. Nat Rev Drug Discov 2022;21:115-40. [Crossref] [PubMed]
Cite this article as: Horn P, Tacke F. Unboxing the cell-type specific contribution of endoplasmic reticulum stress to NASH pathophysiology—myeloid X-box-binding protein 1 as a driver of steatohepatitis and fibrosis. Transl Gastroenterol Hepatol 2023;8:14.